Research points
This article investigates the metabolic mechanism of glutathione (GSH) as an antioxidant in Corynebacterium glutamicum, and constructs an efficient glutathione producing strain through metabolic engineering and fermentation optimization strategies.
Research has found that by modifying glutathione synthase (GshF), knocking out genes related to hydrogen sulfide production and glutathione degradation, and combining with overexpression of glutathione reductase (GR), the production of glutathione can be significantly increased and the redox balance of cells can be maintained.
In a 5 L fermentation tank, a glutathione yield of 25.07 g/L was ultimately achieved through multi parameter optimization.
This study not only demonstrates the potential of Corynebacterium glutamicum in industrial production of glutathione, but also provides effective strategies for addressing oxidative stress in food fermentation processes.
Enzyme modification and optimization
A mutant StGshFE11P-H366Y-A398E with higher catalytic activity and stability was obtained by modifying glutathione synthase (GshF) through the computer-aided design platform Pross, significantly improving the efficiency of glutathione synthesis.
Gene Knockout and Metabolic Engineering
Knocking out the aecD gene related to hydrogen sulfide production and the ggt gene related to glutathione degradation reduced hydrogen sulfide production and glutathione degradation, thereby increasing glutathione production.
Application of glutathione reductase
Overexpression of glutathione reductase (GR) enhances the reducing ability of cells, effectively reduces the accumulation of glutathione disulfide (GSSG), and maintains the redox balance of cells.
Fermentation optimization strategy
In a 5 L fermentation tank, efficient production of glutathione was achieved by optimizing parameters such as substrate addition time, pH value, temperature, and dissolved oxygen. The final yield reached 25.07 g/L, the yield reached 163.86 mg/g glucose, and the production efficiency was 417.83 mg/L/h.
Industrial application potential
The study demonstrated the potential of Corynebacterium glutamicum in industrial production of glutathione, providing an effective chassis cell strategy for solving oxidative stress problems in food fermentation processes and having important industrial application value.
Chart interpretation
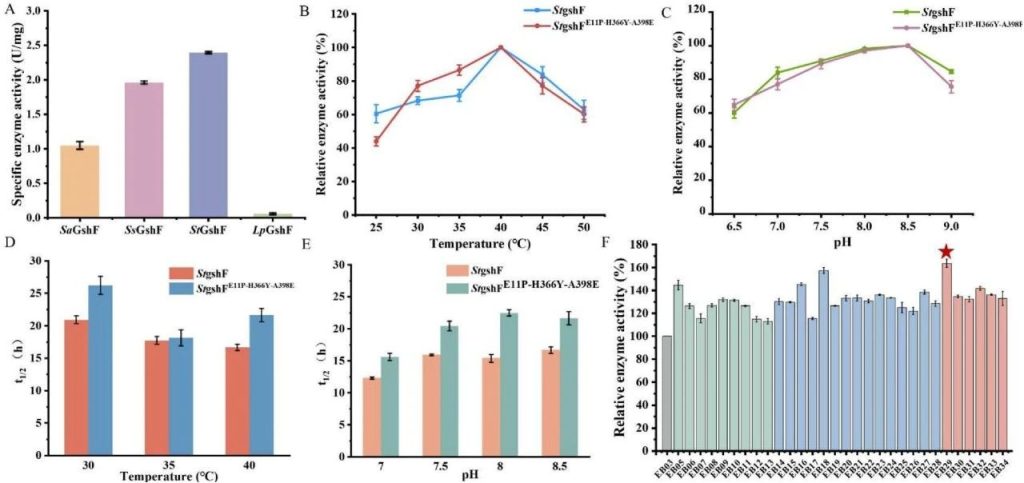
Screening and enzymatic characteristics of GshF enzymes from different sources.
A: The specific enzyme activity of GshF enzymes from different sources. The results showed that the enzyme activity from Streptococcus thermophilus (StGshF) was the highest, at 2.39 U/mg.
B: The relative enzyme activity of StGshF and its mutants at different temperatures. The optimal temperature is 40 ° C.
C: The relative enzyme activity of StGshF and its mutants at different pH values. The optimal pH is 8.5.
D: The half-life (t1/2) of StGshF and its mutants at different temperatures. The mutant StGshFE11P-H366Y-A398E has a t1/2 of 21.6 hours at 40 ° C, which is 29.94% higher than the wild type.
E: The half-life of StGshF and its mutants at different pH values. The t1/2 of the mutant increased to 26.2 hours, 18.1 hours, and 22.5 hours at pH 7.0, 7.5, and 8.0, respectively.
F: Relative enzyme activity of different mutants. The relative enzyme activity of the three mutant StGshFE11P-H366Y-A398E is the highest, at 163.62%.
This figure shows the performance improvement of GshF enzyme screened and modified through the computer-aided design platform Pross.
The mutant not only increased enzyme activity, but also significantly improved thermal stability and pH stability, providing a key enzyme foundation for subsequent fermentation experiments.
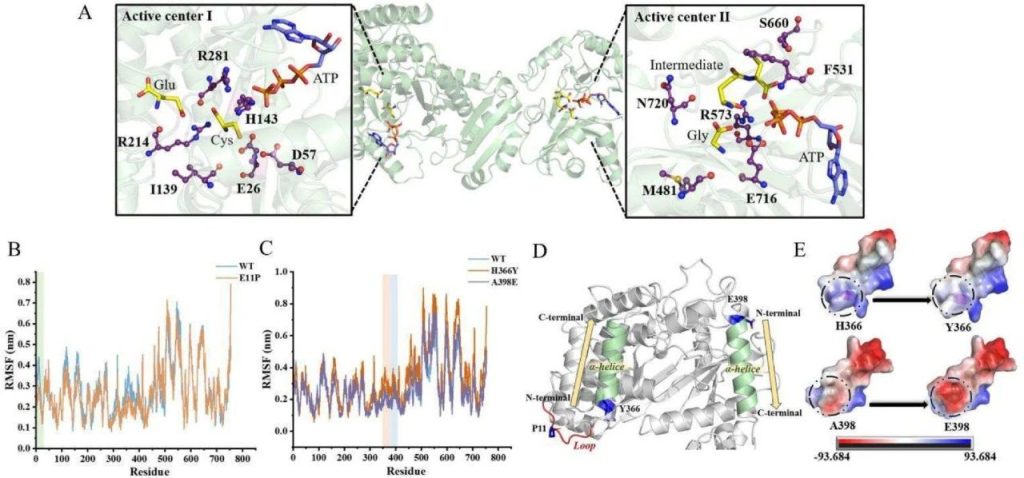
The active sites and catalytic mechanism of GshF enzyme were analyzed through molecular docking and molecular dynamics simulation.
A: Molecular docking results of StGshF with substrates (L-glutamic acid, L-cysteine, L-glycine, and ATP). L-Glutamic acid first binds to L-cysteine and ATP in the active center I region, and then binds to L-glycine and ATP in the active center II region.
B: RMSF (root mean square fluctuation) results of wild-type and E11P mutant. The flexibility of the E11P mutant is significantly reduced, indicating an increase in rigidity in this region, which helps to improve enzyme stability.
C: RMSF results of wild-type, H366Y, and A398E mutants. The fluctuation of H366Y and A398E is not significant, indicating that the mutations at these sites have a relatively small impact on the stability of the enzyme.
D: The location of the mutation site in the StGshF protein. E11 is located on the circular structure of the protein, while H366 and A398 are located at the N-terminus of the alpha helix.
E: Changes in electrostatic potential energy at the transition points of H366Y and A398E. After the mutation, the electrostatic potential energy tends to move away from positive values, indicating that these mutations optimize the stability of the alpha helix.
This figure reveals the catalytic mechanism of GshF enzyme and the effect of mutation sites on enzyme stability through molecular docking and dynamic simulation.
The E11P mutation enhances enzyme stability by increasing rigidity, while the H366Y and A398E mutations improve enzyme thermal stability by optimizing the stability of the alpha helix.
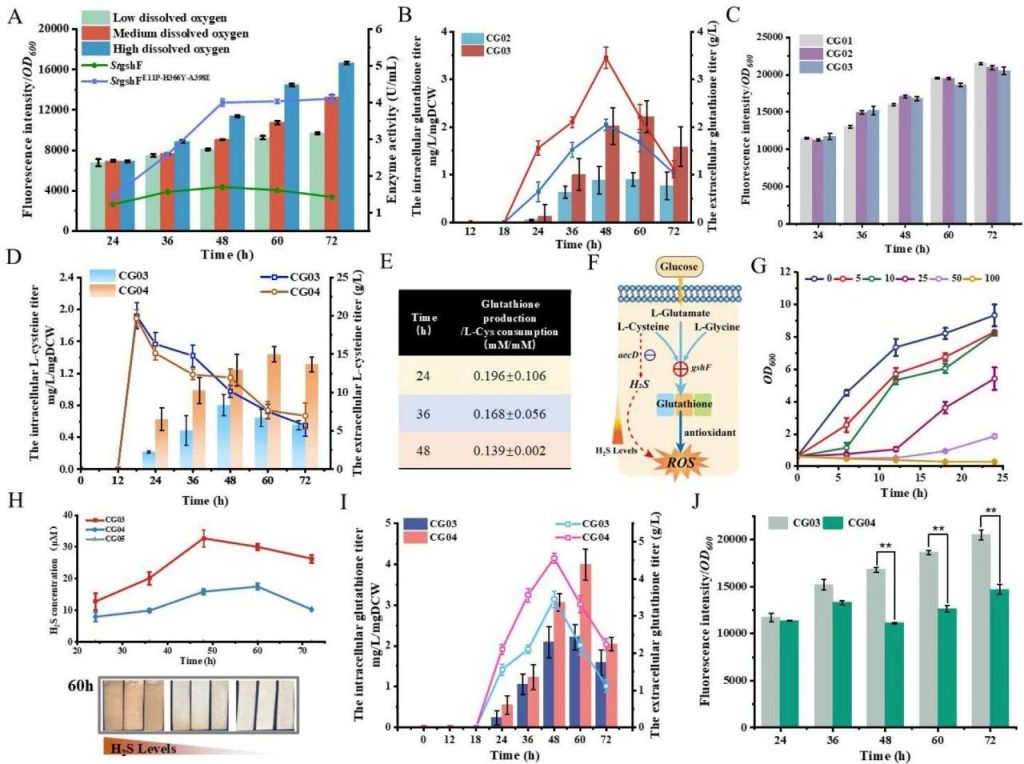
The effects of hydrogen sulfide and glutathione induced by L-cysteine on redox homeostasis.
A: Changes in ROS levels and enzyme activity of StGshF and StGshFE11P-H366Y-A398E under different dissolved oxygen conditions.
The results showed that with the increase of dissolved oxygen, the level of ROS gradually increased, and the enzyme activity of the mutant was significantly higher than that of the wild type.
B: The intracellular and extracellular glutathione production of CG02 and CG03 strains. The extracellular glutathione production of CG03 strain is 3.47 g/L, which is 169.3% higher than that of CG02 strain.
C: Changes in ROS levels during fermentation of CG01, CG02, and CG03 strains. The ROS levels of CG03 strain were significantly higher than those of CG01 strain at 36 and 48 hours.
D: Intracellular and extracellular L-cysteine levels of CG03 and CG04 strains. The intracellular accumulation of L-cysteine in CG04 strain increased, while the degradation rate decreased.
E: The ratio of glutathione production to L-cysteine consumption in CG03 strain. The ratio is only 0.14-0.20, indicating that the metabolism of L-cysteine flows towards other pathways.
F: Schematic diagram of the effects of hydrogen sulfide and glutathione induced by L-cysteine on ROS levels.
G: The effect of different concentrations of hydrogen sulfide on the growth of Corynebacterium glutamicum.
H: The hydrogen sulfide levels of CG03, CG04, and CG05 strains.
I: The intracellular and extracellular glutathione production of CG03 and CG04 strains.
J: Changes in ROS levels during fermentation of CG03 and CG04 strains.
This figure illustrates the process by which L-cysteine affects the redox steady state by generating hydrogen sulfide.
The accumulation of hydrogen sulfide and the metabolic flow of L-cysteine to other pathways lead to a decrease in glutathione production, further exacerbating oxidative stress.
By knocking out the aecD gene, the production of hydrogen sulfide was reduced, glutathione production was increased, and ROS levels were reduced.
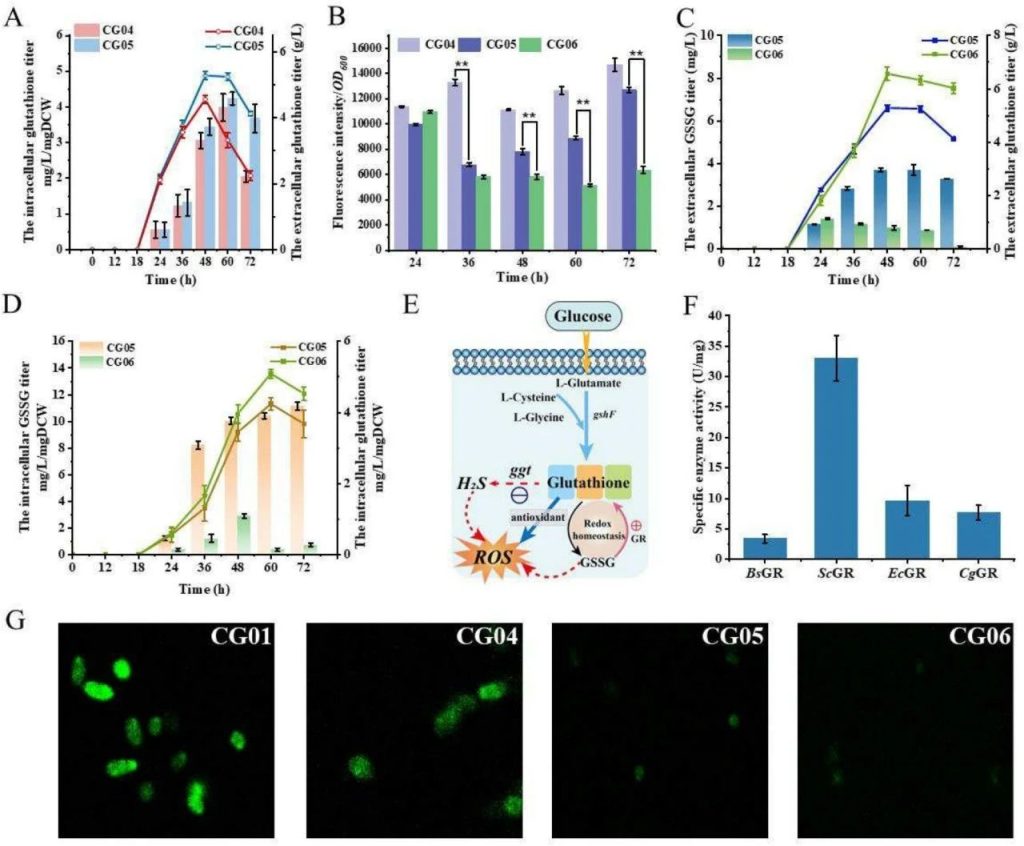
The effects of hydrogen sulfide and GSSG on ROS levels.
A: The intracellular and extracellular glutathione production of CG04 and CG05 strains. The extracellular glutathione production of CG05 strain is 5.28 g/L, which is 21.97% higher than that of CG04 strain.
B: Changes in ROS levels during fermentation of CG04, CG05, and CG06 strains. The ROS level of CG06 strain is significantly lower than that of CG05 strain.
C: The extracellular GSSG and glutathione production of CG05 and CG06 strains. The extracellular GSSG of CG06 strain was significantly reduced.
D: The intracellular GSSG and glutathione production of CG05 and CG06 strains. The intracellular GSSG accumulation of CG06 strain decreased.
E: Schematic diagram of the effects of hydrogen sulfide and GSSG on ROS levels.
F: The specific enzyme activity of glutathione reductase (GR) from different sources. The GR activity from brewing yeast is the highest.
G: The intracellular ROS fluorescence changes of CG01, CG04, CG05, and CG06 strains at 60 hours.
This figure shows that knocking out the ggt gene and overexpressing glutathione reductase (GR) can reduce the accumulation of GSSG, increase glutathione production, and significantly reduce ROS levels.
This indicates that metabolic engineering can effectively improve the redox state of cells.
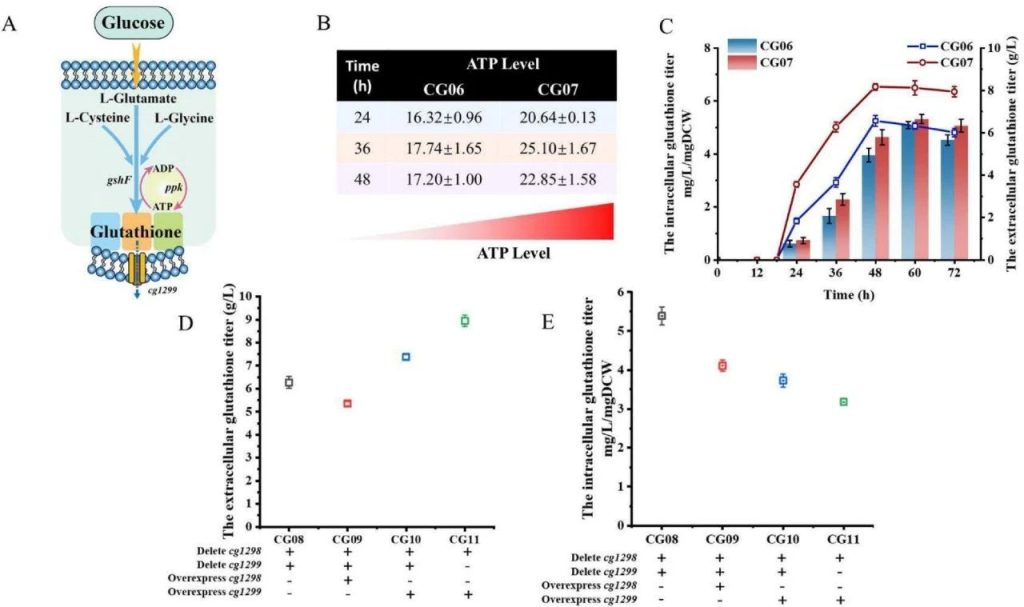
The effect of energy metabolism and transport system on the antioxidant capacity of Corynebacterium glutamicum.
A: Schematic diagram of energy regeneration and glutathione transport.
B: The intracellular ATP levels of CG06 and CG07 strains at 24, 36, and 48 hours. The intracellular ATP levels of CG07 strain increased by 29.25%, 43.14%, and 32.62% compared to CG06 strain.
C: The intracellular and extracellular glutathione production of CG06 and CG07 strains. The extracellular glutathione production of CG07 strain is 8.17 g/L, which is 24.54% higher than that of CG06 strain.
D: The extracellular glutathione production of CG08, CG09, CG10, and CG11 strains. The extracellular glutathione production of CG11 strain is 8.95 g/L, which is 42.74% higher than that of CG08 strain.
E: The intracellular glutathione production of CG08, CG09, CG10, and CG11 strains. The intracellular glutathione production of CG11 strain is 3.18 mg/L/mgDCW, which is lower than that of CG08 strain.
This figure shows that overexpression of PPK enzyme increases intracellular ATP levels, and knocking out cg1298 and overexpression of cg1299 genes enhances glutathione transport efficiency, resulting in a significant increase in glutathione production.
This indicates that optimizing energy metabolism and transport systems is an important strategy for increasing glutathione production.
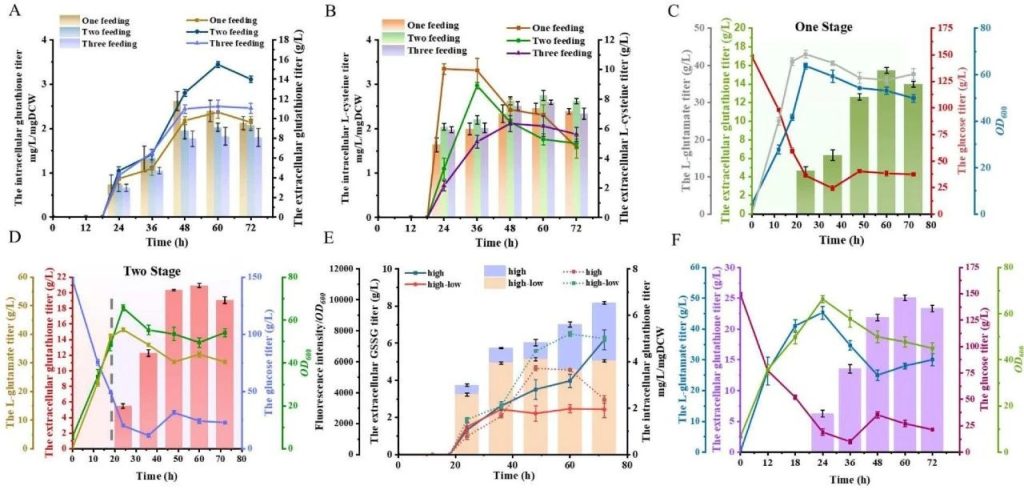
The effect of multi parameter collaborative fermentation on glutathione production of CG11 strain.
A: The intracellular and extracellular glutathione production of CG11 strain under different substrate addition times. When adding substrates in two steps, the extracellular glutathione production was the highest, reaching 15.48 g/L.
B: Intracellular and extracellular L-cysteine levels of CG11 strain under different substrate addition times. Two step substrate addition can reduce the oxidation of L-cysteine.
C: Glucose levels of CG11 strain under single-stage fermentation conditions OD600、 Extracellular glutathione production and L-glutamate levels.
D: Glucose levels of CG11 strain under two-stage fermentation conditions OD600、 Extracellular glutathione production and L-glutamate levels. The extracellular glutathione production of two-stage fermentation was 20.92 g/L, which was 35.14% higher than that of single-stage fermentation.
E: Extracellular GSSG levels, intracellular glutathione levels, and ROS levels of CG11 strain under different dissolved oxygen conditions. Under low dissolved oxygen conditions, intracellular glutathione accumulation increases and ROS levels decrease.
F: Glucose levels of CG11 strain under low dissolved oxygen conditions OD600、 Extracellular glutathione production and L-glutamate levels. The final glutathione production reached 25.07 g/L.
This figure shows that by optimizing substrate addition time, fermentation temperature and pH value, as well as controlling dissolved oxygen levels, the production of glutathione can be significantly increased.
The optimization strategy under two-stage fermentation and low dissolved oxygen conditions effectively reduced oxidative stress and increased glutathione accumulation.
Inspiration and Reflection
1. The Importance of Redox Balance
This article significantly increased glutathione production and maintained cellular redox balance by knocking out the aecD and ggt genes, as well as overexpressing glutathione reductase (GR).
This indicates that maintaining the redox balance of cells is the key to increasing the yield of target products in industrial fermentation.
In the industrial fermentation process, more attention should be paid to the redox state of cells and optimized through metabolic engineering methods to improve the yield of target products and the robustness of cells.
2. Dynamic regulation of metabolic pathways
This article optimized the metabolic pathway of glutathione by knocking out and overexpressing specific genes.
In the future, dynamic regulatory strategies can be further explored, such as real-time adjustment of the expression levels of key enzymes in metabolic pathways through sensors or feedback control systems, to increase glutathione production.
Develop an intelligent fermentation system that utilizes biosensors and automation control technology to achieve dynamic regulation of metabolic pathways.
3. Exploring new redox regulatory factors
This article enhances the reducing ability of cells by overexpression of glutathione reductase (GR).
In the future, other redox regulatory factors such as NADH/NAD+, NADPH/NADP+, etc. can be further explored to optimize the redox state of cells.
Through genomics and transcriptomics analysis, search for new redox regulatory factors and optimize them through metabolic engineering methods.
4. Synthesis of Glutathione from Whole Glucose
This article improved the production of glutathione by optimizing metabolic pathways. In the future, further exploration can be conducted on how to fully synthesize glutathione from glucose and reduce dependence on exogenous precursors.
By using metabolic engineering methods to optimize the glucose metabolism pathway and improve the supply efficiency of precursor substances such as L-glutamate, L-cysteine, and L-glycine, complete synthesis of glutathione from glucose can be achieved.
Concept learning
1. Redox Balance
Redox equilibrium is a dynamic state of balance between the oxidized and reduced substances within a cell, which is crucial for maintaining normal physiological functions of the cell.
The intracellular redox state is mainly maintained by redox pairs such as NADH/NAD ⁺, NADPH/NADP ⁺, glutathione (GSH)/glutathione disulfide (GSSG).
These redox reactions play a crucial role in cellular metabolism, signal transduction, and antioxidant defense.
Glutathione system
Glutathione is one of the most important antioxidants in cells, protecting cells from oxidative damage by directly clearing free radicals and reactive oxygen species (ROS).
Glutathione reductase (GR) can reduce oxidized GSSG to reduced GSH, thereby maintaining cellular redox balance.
In industrial fermentation, maintaining the balance of the glutathione system is crucial for improving the robustness of cells and the yield of target products.
NADH/NAD ⁺ and NADPH/NADP ⁺ systems
These two systems play a central role in the energy metabolism and synthesis metabolism of cells.
NADH mainly participates in energy production in the cellular respiratory chain, while NADPH provides reducing power for synthetic metabolism.
By optimizing the balance of these redox pairs, the metabolic efficiency of cells and the ability to synthesize target products can be improved.
Systematic regulation of redox equilibrium
This article significantly increased glutathione production and cellular antioxidant capacity by knocking out the aecD and ggt genes, as well as overexpressing GR.
However, redox balance is a complex system involving multiple metabolic pathways and regulatory factors.
In the future, it is necessary to conduct more comprehensive research on the redox status of cells and construct more complete metabolic models through systems biology methods to achieve more efficient regulation of redox balance.
2. Metabolic Engineering
Metabolic engineering is the modification of microbial metabolic pathways through genetic manipulation to increase the yield of target products and cellular performance.
The core of metabolic engineering lies in the systematic analysis and optimization of metabolic networks, including the regulation of enzyme activity, reconstruction of metabolic pathways, and regulation of cellular physiological states.
Enzyme Engineering
Through genetic engineering and protein engineering methods, key enzymes are modified to enhance their catalytic activity, stability, and substrate specificity.
For example, in this article, the computer-aided design platform Pross was used to modify glutathione synthase (GshF), resulting in mutants with higher catalytic activity and stability. This enzyme engineering strategy not only improves the synthesis efficiency of glutathione, but also enhances the robustness of cells in industrial fermentation.
Metabolic Flux Analysis (MFA)
By quantitatively analyzing the flux distribution in the metabolic network, metabolic bottlenecks and key nodes can be identified, providing theoretical basis for metabolic engineering.
For example, by knocking out the aecD and ggt genes, the production of hydrogen sulfide and degradation of glutathione were reduced, optimizing the metabolic flux of glutathione.
systems biology
Combining multiple omics technologies such as genomics, transcriptomics, proteomics, and metabolomics, comprehensively analyze the metabolic network and regulatory mechanisms of cells.
Systems biology methods can help researchers gain a deeper understanding of the physiological state of cells and provide more comprehensive guidance for metabolic engineering.
3. Fermentation Optimization
Fermentation optimization is the process of increasing the yield and fermentation efficiency of the target product by adjusting fermentation conditions such as temperature, pH value, dissolved oxygen, substrate addition, etc.
The key to fermentation optimization lies in finding the optimal combination of fermentation parameters to achieve efficient cell growth and metabolism.
Multi parameter collaborative optimization
By systematically adjusting multiple fermentation parameters, the optimal state of cellular metabolism can be achieved.
For example, in this article, efficient production of glutathione was achieved by optimizing parameters such as substrate addition time, pH value, temperature, and dissolved oxygen.
This multi parameter collaborative optimization strategy can significantly improve the yield and fermentation efficiency of the target product.
Dynamic control strategy
By monitoring and regulating key parameters during the fermentation process in real-time, dynamic optimization can be achieved.
For example, by utilizing biosensors and automated control systems, fermentation conditions can be adjusted in real-time to cope with changes in cell metabolism and interference from environmental factors.
Dynamic regulation strategies can further improve the stability and efficiency of the fermentation process.
Scalability of industrial fermentation
After achieving success on a laboratory scale, it is necessary to consider the scalability of the fermentation process to achieve industrial scale production.
This includes the design of fermentation tanks, control of amplification effects, and stability of the process.
Future research needs to focus more on the scalability of fermentation processes and the feasibility of industrial applications.
4. Oxidative stress
Oxidative stress refers to a state in which the production of reactive oxygen species (ROS) within a cell exceeds its antioxidant capacity, leading to cellular damage.
Oxidative stress not only affects the normal physiological functions of cells, but also reduces the production of target products and the robustness of cells.
Therefore, maintaining the redox balance of cells and reducing oxidative stress are key to improving industrial fermentation efficiency.
Antioxidant defense system
Cells resist oxidative stress through various antioxidant enzymes (such as superoxide dismutase, catalase, glutathione reductase, etc.) and antioxidants (such as glutathione, vitamin C, vitamin E, etc.).
Enhancing the antioxidant defense system of cells through metabolic engineering can improve their survival ability and target product production under oxidative stress conditions.
Oxidative stress and metabolic coupling
Oxidative stress not only affects the antioxidant defense system of cells, but also has a profound impact on the metabolic network of cells.
For example, oxidative stress can alter the energy metabolism pathways of cells, affecting the balance of NADH/NAD ⁺ and NADPH/NADP ⁺.
By studying the coupling mechanism between oxidative stress and metabolism, more effective metabolic engineering strategies can be developed to improve cell robustness and target product yield.
The signaling mechanism of oxidative stress
Oxidative stress can activate various signaling pathways within cells, thereby regulating cellular antioxidant responses and metabolic reprogramming.
In the future, it is necessary to conduct more in-depth research on the signaling mechanisms of oxidative stress, develop new metabolic engineering strategies, and enhance cellular antioxidant capacity and target product production by regulating these signaling pathways.